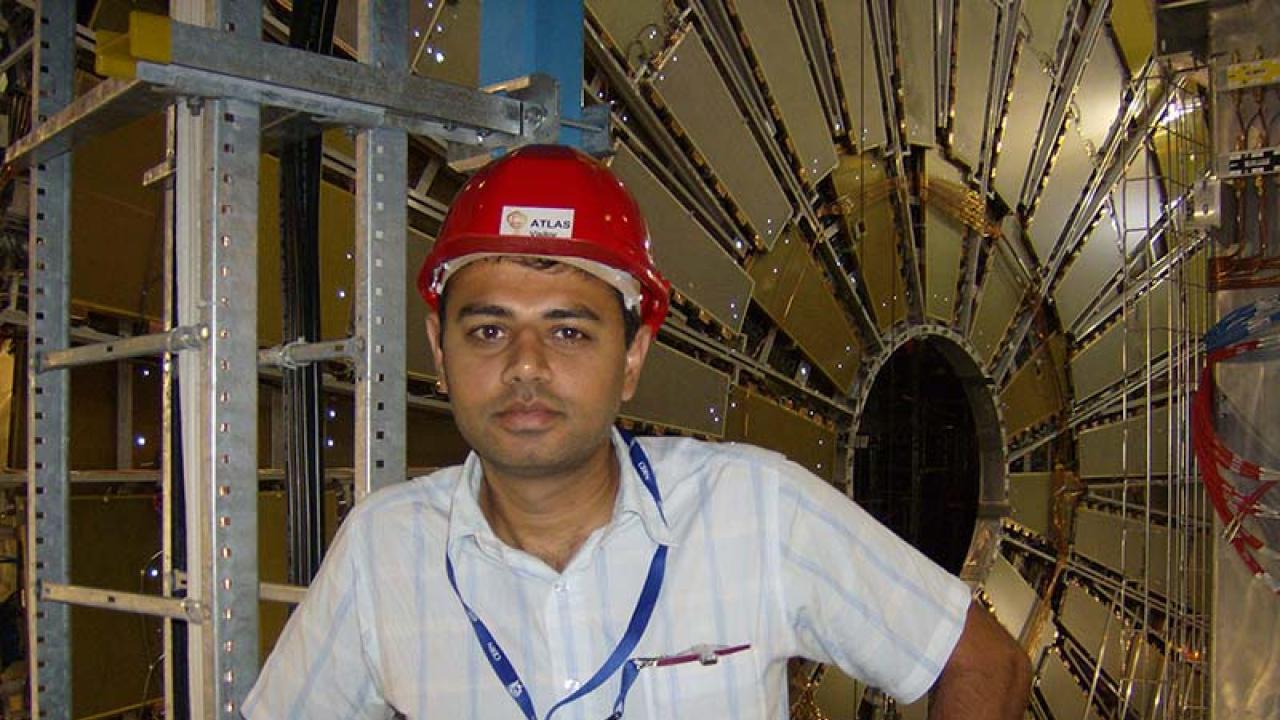
On 14 March 2013, two collaborating experiments (ATLAS and CMS) at the Large Hadron Collider (LHC) announced their new results that reconfirmed the findings presented at CERN in July 2012: the particle discovered last year is very likely the Higgs boson. But what makes this announcement important? How much more do we know about the particle today than we did last year? What further aspects will the researchers be looking at?
Bobby Acharya, a research scientist with ICTP's High Energy, Cosmology and Astroparticle Physics section breaks down the details of the new results and their significance. Acharya heads ICTP's contribution to the ATLAS experiment, working in collaboration with the University of Udine under the umbrella of the Italian National Institute of Nuclear Physics (INFN).
How much more do we know about the particle, that is most likely the Higgs boson, today as compared to July last year? If we have more information, why is that so?
There are two basic reasons: more data and more detailed measurements of the properties of the particle.
We have more data because the running conditions of the LHC were different in 2011 and 2012. There were about five times as many collisions in 2012 compared to 2011. In 2011, the LHC was making around 100 million proton-proton collisions per second and on average only one Higgs boson was produced every 10 seconds. In 2012, out of the 1 billion collisions per second, one Higgs boson was produced every second. The upshot is that in 2011 we produced around 100,000 Higgs bosons and in 2012 we had almost one million. Now, that sounds like a lot. However, we don't directly detect the Higgs boson. It decays very quickly (in a millionth of a billionth of a billionth of a second) into lighter Standard Model particles, some of which we will detect directly and measure and then infer the properties of the Higgs.
Roughly 80 percent of the decays of the Higgs are into final state combinations (e.g., a bottom quark pair or a pair of gluons) that are almost impossible to dig out from the data because those sorts of final states are produced tens of millions of times more frequently by other Standard Model processes not involving the Higgs boson at all. So we say that those final states of the Higgs are "swamped by background".
About 20 percent of the time the Higgs decays into a pair of W-bosons (the particles originally predicted by Salam and Weinberg). But, W-bosons are also unstable. About five percent of the time though, a pair of W-bosons will decay into a final state that includes an electron-positron pair, a muon-antimuon pair, an electron-antimuon pair or a positron-muon pair; these are produced together with neutrinos. These are the final states that are not completely swamped by background. However, the neutrinos in the event, being neutral and weakly interacting, fly out of the detector and their presence is inferred as "missing energy", but they carry with them vital information about the event (and hence information of the Higgs boson from which they have originated). Since these neutrinos fly out, it is challenging to infer the properties of the particle that produced them. Also, five percent of 20 percent is only one percent -- so there were around 1,000 of these events in 2011 and something like 5,000 in 2012. Similarly, around five percent of the time the Higgs decays to two Z-bosons (again predicted by Salam and Weinberg). Of these, a small fraction produces events with four charged electron/positron/muon/antimuon combinations, which are not swamped by background. There are no neutrinos in these events, so we can reconstruct the Higgs completely in these cases. In 2011, we had about 10 events total compared to 25 or so in 2012. Finally, around one in 500 Higgs bosons decays to two photons. Even though this is rare, we can understand the background well enough to see it. We had about 200 events in 2011 and almost 1,000 in 2012. Again, these events have no neutrinos so, if we measure the energies and momenta of the photons precisely enough, we can infer properties of the Higgs such as its mass and spin in principle.
Now, coming to more detailed measurements: According to the Standard Model, all particles, except the Higgs boson, should have a definite, non-zero spin in space, i.e., they are literally rotating (albeit in discrete, fixed amounts). The Higgs boson should not have any such spin, but we have to prove that. Further, the decays of the Higgs boson should not violate parity (a property that relates to the symmetry of the wave function representing a system of fundamental particles). So, if we looked at a mirror image of the decays of Higgs bosons we would not notice any difference. In July last year we didn't have enough events to start discriminating the spin and parity properties of the Higgs with much confidence. The increased data that the LHC delivered between July and the end of 2012 as well as some more sophisticated analysis ideas have allowed us to say with much more confidence that the new particle is actually a Standard Model Higgs boson!
What are some other aspects that the physicists will be looking at to confirm that the particle is indeed the Higgs boson?
We will try and develop even more sensitive measurements of the spin and parity. Also, the Higgs -- since it is responsible for the masses of Standard Model particles -- interacts with all particles. It is very important for us to measure ALL of these interactions. At the moment, for instance, we don't have very sensitive measurements of the interaction of the Higgs with fermions, i.e., quarks and leptons. All the measurements mentioned above are when the Higgs decays to vector bosons, (W's Z's and photons). For the interactions with fermions, we need more data so will have to wait until the LHC starts up again in 2015.
Could you explain briefly why it is important to understand if the Higgs boson fits into the Standard Model or goes beyond the Standard Model? What are some of the challenges in understanding where exactly the particle fits?
This is of paramount importance! The Higgs - being the giver of mass - couples directly to all particles with mass and even indirectly to all particles without mass (e.g., photons). So, if there is physics beyond the Standard Model, then we expect some properties of the Higgs to be different with respect to the Standard Model. For instance, there could be new particles, which are light enough for the Higgs to decay into them. An example could be new neutral particles, which are dubbed "invisible Higgs decays" since the neutral particles would escape detection like the neutrinos. Actually, our Udine/ICTP ATLAS group is working on invisible Higgs decays and we aim to have a new ATLAS paper ready on this in the summer. Loan Truong (a former ICTP diploma student from VietNam and now a SISSA/ICTP PhD student) and I are working on this together with ATLAS physicists from Brookhaven National Laboratory, University of Texas at Arlington, University of Wisconsin and University of Montreal. But in general, we will look for "excesses" or "deficits" in the number of Higgs decays into a given final state with respect to the Standard Model.